Millifluidic
Millifluidic is a branch of fluid dynamics that emerges as a captivating frontier in science and engineering, distinguished by its unique advantages to improve the reliability of complex biological models by incorporating dynamic characteristics typical of physiological systems.
The Genesis and Evolution of Millifluidic
The origins of millifluidic can be traced back to early explorations in fluid mechanics and the need for precise fluid control in various scientific and industrial applications. Despite its miniature scale, millifluidics offers distinct benefits and solutions, particularly within biomedical applications. This innovative field is shaping the future of biotechnology, enabling groundbreaking discoveries and advanced research methodologies.
In the realm of biomedical applications, millifluidics plays a significant role in translational research, drug discovery, and personalized medicine.
The Journey Forward
As the field continues to evolve, millifluidics promises to drive further innovations in biotechnology. Researchers are exploring new materials and fabrication techniques to create more sophisticated and versatile millifluidic devices.
The impact of millifluidics on research is profound, providing researchers with robust tools to explore complex biological systems, model human diseases, and develop new therapeutic strategies.
Its potential to accelerate scientific discoveries and enhance the efficiency of biomedical research makes it a critical area of focus in modern biotechnology.
Why use a Millifluidic platform in biomedicine:
In biomedicine, millifluidics refers to the manipulation of fluids at the millimeter scale, which is crucial for various scientific and industrial applications. Millifluidics offers several advantages, such as:
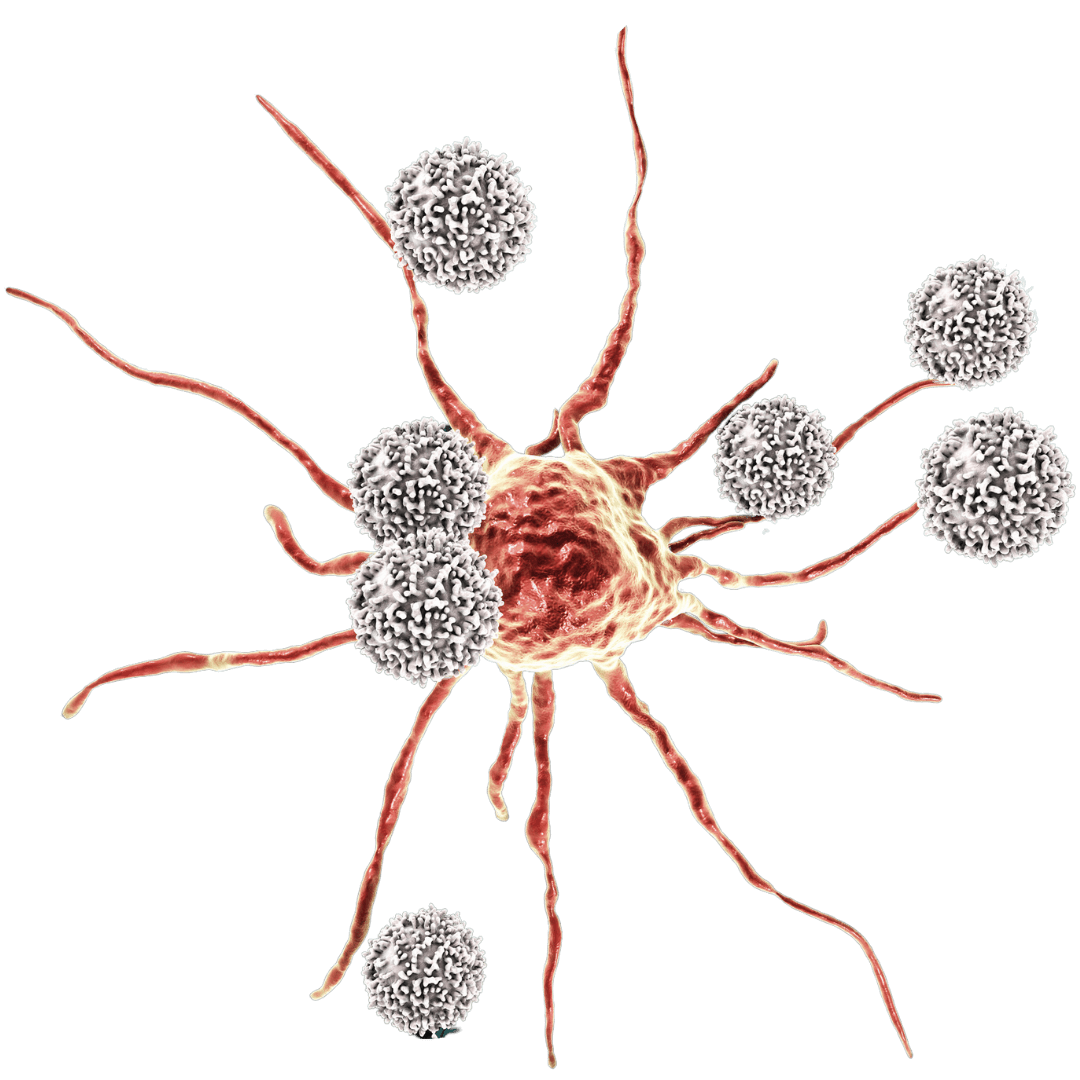
Differences between Millifluidic and Microfluidic in Biomedical Applications
In millifluidics, fluids are manipulated and observed within channels ranging in size from millimeters. Although larger quantities of liquids are used compared to microfluidics, these amounts remain minimal compared to tests conducted using traditional methods such as Petri dishes and flasks.
The need to miniaturize volumes within microfluidic devices stems from diagnostics, where small amounts of biological liquids are manipulated and interact with expensive reagents. However, these benefits do not directly translate to translational research and late-stage drug discovery. Indeed, miniaturization is not imperative for achieving physiological relevance and predictive models. An optimal scale for such models is closer to the millimeter size.
Millifluidic systems aim to simulate the physiological environment of human organs more accurately, enabling the study of complex biological processes in vitro. They are particularly valuable for replicating the mechanical and fluidic conditions of human tissues, making them indispensable for drug testing, disease modeling, and personalized medicine applications.
In the area of microphysiological systems (MPS), a millifluidic device is a scaled platform specifically designed for manipulating and studying fluids at the milliliter scale, typically ranging from hundreds of microliters to a few milliliters. These devices incorporate channels and chambers that are generally larger than those found in microfluidic systems. This larger size allows for the culture of clinically relevant-sized cellular models, while maintaining and observing cellular and tissue constructs under precisely controlled flow conditions.
Millifluidics is instrumental in improved understanding of cellular behaviors in various physiological conditions by adopting organ-on-a-chip models, which mimic human physiology and disease conditions.
The use of millifluidic devices addresses practical challenges in cellular cultures by providing a platform that enables more physiologically relevant nutrient diffusion.
This aspect is crucial for achieving predictive models and replicating biological mechanisms accurately.
Millifluidic devices offer a solution to these challenges by allowing for precise control over flow conditions and nutrient delivery. Research by Dahl et al. (2015) has demonstrated the capability of millifluidic systems to maintain stable and controlled shear flow conditions, which are essential for ensuring optimal nutrient transport within cellular cultures. By mimicking the physiological environment more accurately, millifluidic devices promote cell viability and function, thus enabling the development of more predictive in vitro models.
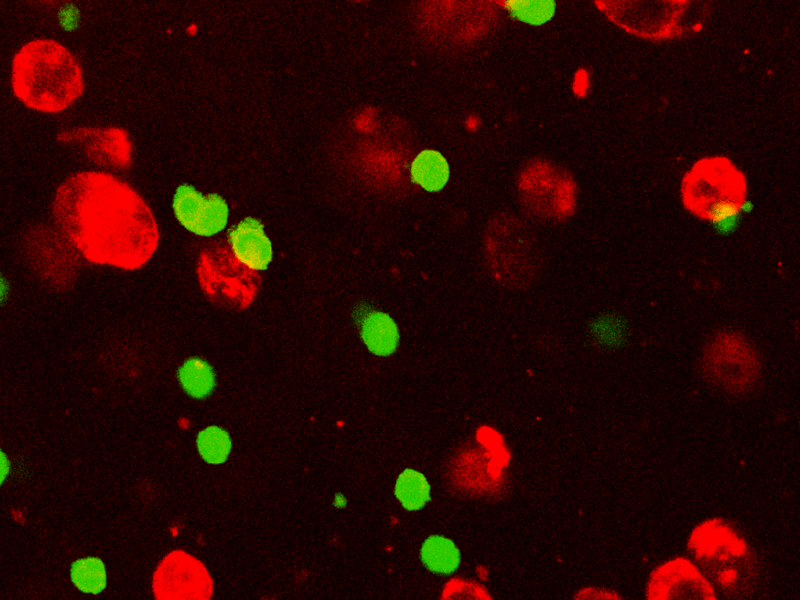
Key applications of millifluidic in biomedicine
Cell Culture
Millifluidics provides a controlled microenvironment for the culture and analysis of cells, tissues, and organoids.
Millifluidic platforms, like organ on chips, are used for resembling in vitro cell behavior, tissue development, drug responses, and disease mechanisms, offering insights into various biomedical processes. These devices enable precise manipulation of culture conditions such as nutrient supply, oxygenation, and mechanical stimulation, allowing researchers to mimic physiological conditions more accurately than static conditions.
Drug discovery
Millifluidic systems are employed in drug discovery and development processes, including high-throughput screening, pharmacokinetic studies, and drug formulation optimization.
These devices enable researchers to miniaturize and automate various experimental procedures, accelerating the identification of lead compounds, elucidation of drug mechanisms, and optimization of drug formulations. Millifluidic-based approaches offer advantages such as reduced reagent consumption, faster experimental turnaround times, and enhanced experimental reproducibility.
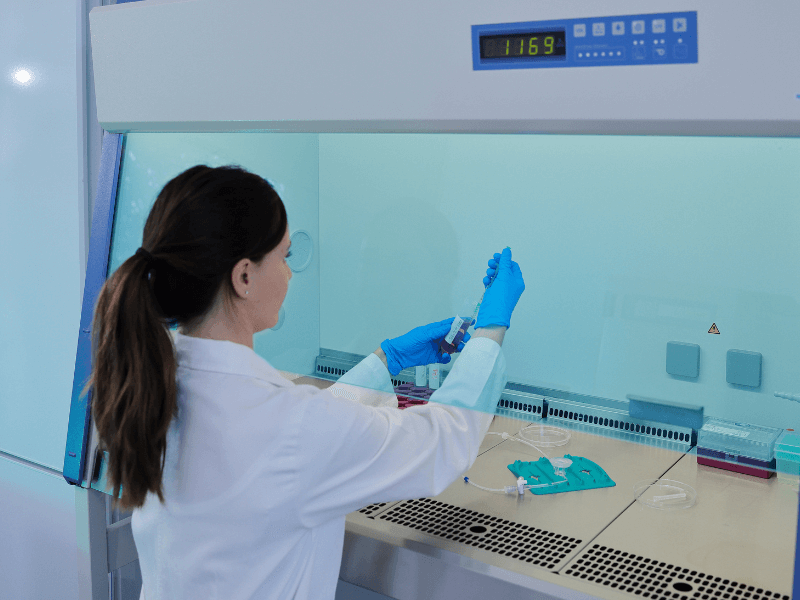
Organ-on-chip models
Millifluidic organ-on-chip models replicate the structure and function of human organs in vitro, providing platforms for studying organ physiology, disease mechanisms, and drug responses.
These devices integrate fluidic channels, cell culture chambers, and functional components to recreate the complexity of human organs in a controlled environment. Millifluidic organ-on-chip models have applications in drug screening, toxicity testing, disease modeling, and personalized medicine, offering insights into organ-level responses that cannot be captured using traditional cell culture or animal models.
This larger size allows for the maintenance and observation of cellular and tissue constructs under precisely controlled flow conditions.
Studies such as those conducted by Bhargava, Thompson, & Malmstadt (2014) highlight the importance of fluid properties, including inertial force and viscous force, in governing nutrient transport within millifluidic systems. The study of these parameters allows researchers to design and optimize millifluidic devices for efficient nutrient diffusion, thereby supporting the long-term viability of tissue cultures.
The forces experienced within millifluidic devices are primarily governed by fluid properties such as inertial force, viscous force, and fluid interfacial properties (Bhargava, Thompson, & Malmstadt, 2014). These devices offer highly controlled shear flow conditions, which have been extensively studied and documented over the past three decades (Dahl et al., 2015). As a result, they are often utilized for shear and viscosity measurements. However, they also find applications in particle focusing, separation and transport, as well as phase transitions of polymeric materials.
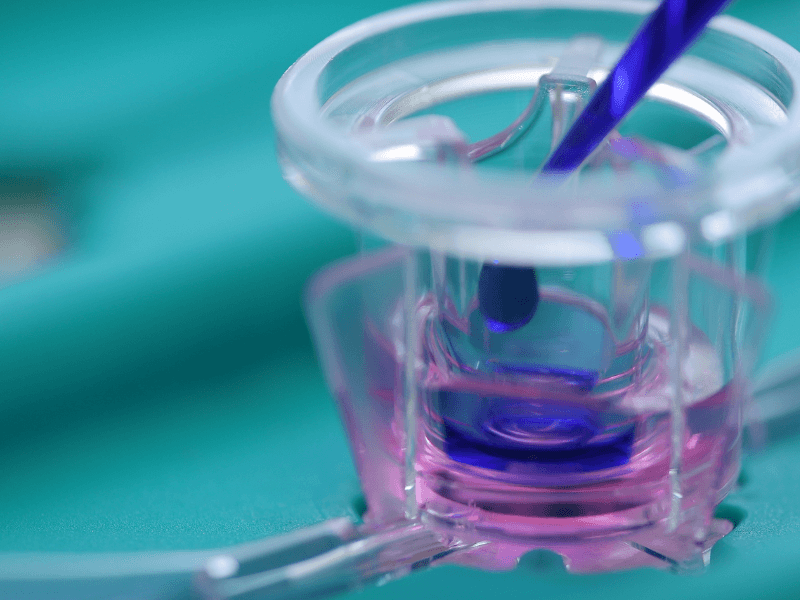
Physiological flow velocities:
Millifluidic systems allow for the setting of various physiological flow rates, which can differ significantly from one another.
Millifluidic Organ On Chip Benefits
Millifluidic cell culture platforms offer significant advantages for biomedical research, enhancing the physiological relevance and efficiency of in vitro studies. These platforms facilitate improved mass transport, more accurate drug diffusion, accelerated cell differentiation, and support for long-term culture.
Moreover, millifluidic enable to:
Increased Mass Transport
The MIVO® Millifluidic platform used in this application, significantly improved the delivery of oxygen and nutrients to cells while effectively removing waste products, closely replicating in vivo conditions. Marrella et al. (2020) also demonstrated that dynamic cultures using millifluidics maintain cellular viability and function more effectively than static cultures. Notably, these figures show that the structure of villi and microvilli is preserved under dynamic conditions, whereas these structures deteriorate in static environments.
Marrella, A. (2020) “In vitro demonstration of intestinal absorption mechanisms of different sugars using 3D organotypic tissues in a fluidic device”, ALTEX – Alternatives to animal experimentation.
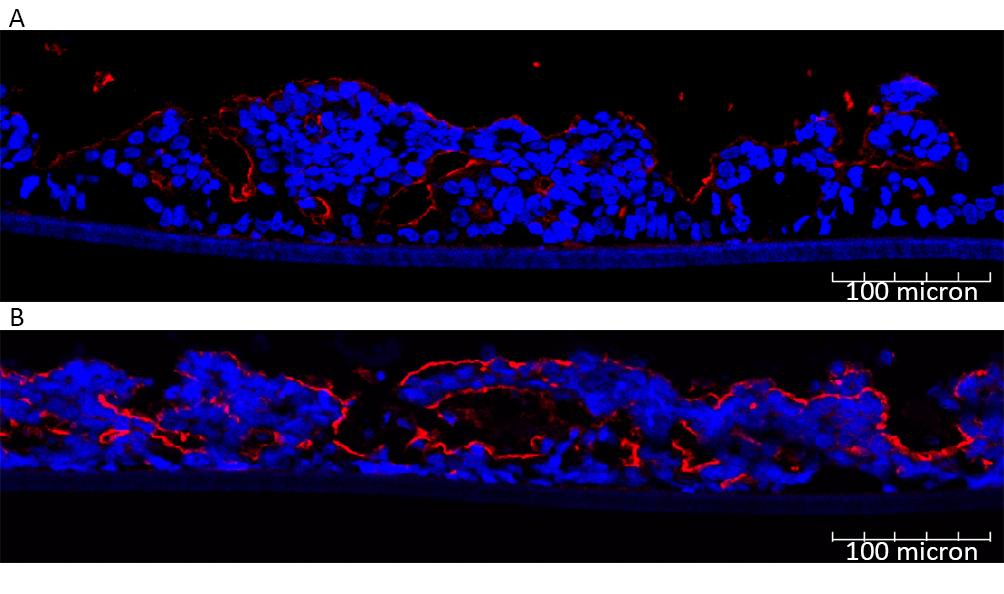
Comparison of villin expression at the apical surfaces of intestinal tissues cultured under static (A) or dynamic (B) conditions in the MIVO® device. From Marrella A, et al. (2020), published under CC BY 4.0.
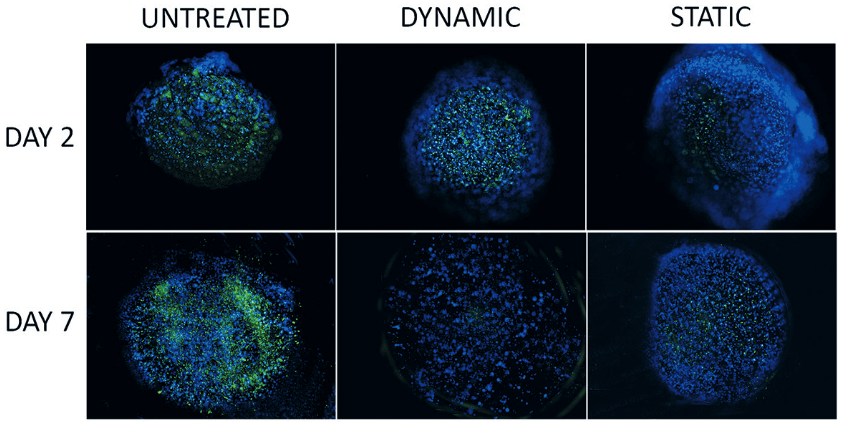
Fluorescence images showing immunostaining of Ki67 (green) as marker of proliferation of SKOV-3 cultured within alginate hydrogels treated with 10 μM cisplatin in static or dynamic conditions (MIVO®). From Marrella A, et al. (2021), published under CC BY 4.0.
More Accurate Drug Diffusion
Millifluidic systems offer a more physiological environment for drug diffusion within 3D tissues. Studies, such as those by Sung and Shuler (2009) and Marrella et al (2021), have shown that drug diffusion in these platforms is highly predictive of in vivo scenarios, providing more reliable data than static culture methods.
Marrella, A. (2021) “3D fluid-dynamic ovarian cancer model resembling systemic drug administration for efficacy assay”
Sung and Shuler (2009) “A micro Cell Culture Analog (micro CCA) with 3-D hydrogel culture of multiple cell lines to assess metabolism-dependent cytotoxicity of anticancer drugs”
Accelerated Cell Differentiation
The dynamic flow conditions in millifluidic devices promote faster and more efficient cell differentiation. Chandorkar P et al. (2017) found that epithelial junctions are tighter under flow conditions, indicating enhanced tissue maturation and functional integrity compared to static conditions.
Chandorkar P et al. (2017) “Fast-track development of an in vitro 3D lung/immune cell model to study Aspergillus infections.”
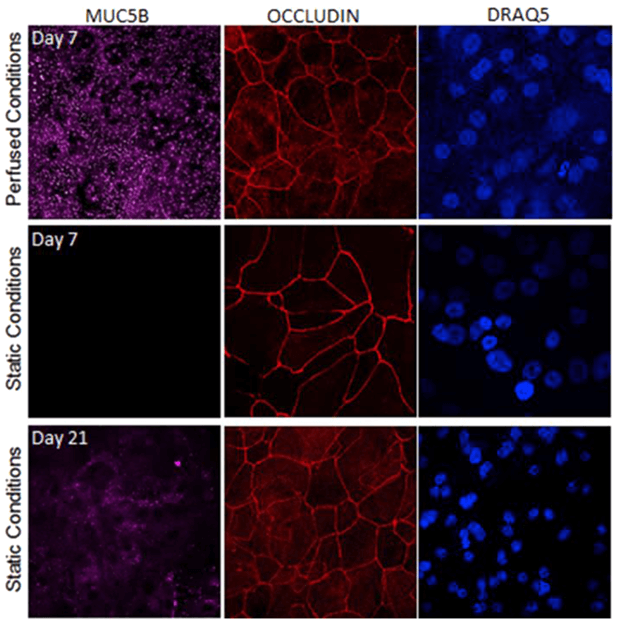
Membrane integrity of respiratory cells under perfused conditions: epithelial cells cultured in dynamic conditions were fully differentiated on day 7 (high amounts of mucus production (lilac), and well-developed tight junctions (red)), while under static conditions no mucus was produced at all on day 7 (middle panel). From Chandorkar P et al. (2017), published under CC BY 4.0
Technical Information:
Comparison between millifluidic and microfluidic
Bubbles
Phenomena such as bubble formation, evaporation and nutrient depletion, are far more difficult to solve at the microscale volumes (i.e. lab on chip), since resistance to flow through a fine channel is inversely proportional to the fourth power of the tube radius r4 (Poiseuille’s law) so a small capillary tube of diameter 200μm will have a resistance per unit length 625 times higher than a tube of 1 mm diameter (Wilkinson, 2023).
Fluid flow in microfluidic channels is particularly difficult to optimize and leads to failure of many organ on chip (OOC) devices. It was Michael Shuler, currently President of Hesperos, who first pointed out that air bubbles are a serious obstacle to a successful operation of a long-term microfluidic systems using cell culture (Sung and Shuler (2009)).
Shear stress
Shear stress plays a crucial role in cellular biology as it significantly influences cell behavior, differentiation, and function. In physiological environments, cells are naturally exposed to shear stress due to blood flow and interstitial fluid movement, which affects processes such as gene expression, protein synthesis, and cellular morphology.
Replicating these dynamic conditions in vitro is essential for creating accurate biological models, particularly for applications like drug testing, disease modeling, and tissue engineering. Therefore, the choice of pumping systems in Organ-on-Chip (OOC) devices is fundamental.
Effective pumping systems ensure consistent and precise fluid flow, providing the necessary shear stress to mimic in vivo conditions. This not only enhances the reliability of experimental results but also enables long-term cell culture and complex tissue formation, making it a cornerstone for advancing biomedical research and applications. If higher pressures are used then there is a risk of rupturing the cell walls of the tissue under culture
Cell culture oxygenation
Another difficulty for microphysiological systems is the lack of medium flow and low oxygen delivered to the cells. There are two simple approaches to solving this problem: the first is to simply increase the radius r of the flow channels, and the second is to increase the pressure. Several commercial OOC developers have taken the latter approach (Emulate, CNBio and TissUse). Unfortunately, this dramatically increases the capital cost and complexity of the control system for the OOC device.
A different strategy has been adopted by Kirkstall and React4life. The chamber and tubing diameters have been increased to millifluidic scale so that low pressures are enough to create adequate flow. Simple peristaltic pumps can then be used. These pumps are low cost and can fit inside a standard cell culture incubator, which is not the case for high pressure pneumatic pumps. The result is a technology which is affordable and yet flexible enough to build multi-organ models.
Different strategies to create the fluid flow in millifluidic
So far, several modes have been adopted for the feeding of these millifluidic stystems, including peristaltic pumps that are mechanically operated and syringe pumps.
For Organ on a Chip devices, the goal should be to limit the pressures that the cells experience to those found in the human body and certainly below those found in large Arteries (diastolic 80 mm of Hg to systolic 120 mm of Hg). These pressures should then guide the selection of pumping mechanisms for use in Organ on a Chip devices. If higher pressures are used then there is a risk of rupturing the cell walls of the tissue under culture.
Syringe pumps use a motorized screw mechanism to move a syringe piston that ensures flows of liquid precisely, in a manner very similar to that of a syringe used for injecting drugs. Peristaltic pumps move liquids using a rotor that gently compresses a tube containing the liquid, producing a regular “pulse” of liquid flow that varies based on the internal volume of the tube, the number of rollers in the rotor and the speed of rotation of the rotor. Peristaltic pumps are more limited in the pressures they can support at around 0.1bar to 0.2 bar (100mm–200 mm of Hg) and these are well within the normal physiological range.
In early Organ on a Chip Research it was hoped that silicon chip based micropumps manufactured by photolithography and using the piezoelectric effect would be effective way to pump medium round flow circuits. However, micropumps were ineffective because they could not overcome the high resistance of microfluidic channels. Many of the OOC developers who originally planned to use micropumps have now migrated to externally driven pneumatic pumps using compressed air or laboratory vacuum lines switched through miniature valves. These valves can be on the chip (for example, TissUse) or on the cartridge that holds the chip (for example, CNBio). Pneumatic pumps can also deliver dangerously high pressure (2 x atmospheric pressure or 2000 mm of Hg) unless there is a pressure monitor in the flow circuit. Although not strictly a pump, Rocker Plates are also used to induce flow under gravity and the equivalent pressure is 0.001–0.01 atmospheric (1–10 mm of Hg). If this type of gravity driven flow device is connected to microfluidic channels with high resistance the levels of flow will be very limited.
Need more information or help to set up your millifluidic experiment?
If you have any questions or need further information about millifluidics and its applications, please don’t hesitate to contact us. We are here to help you explore the potential of this innovative technology. Feel free to reach out to us at info@millifluidic.com.
- The Genesis and Evolution of Millifluidic
- The Journey Forward
- Why use a Millifluidic platform in biomedicine:
- Differences between Millifluidic and Microfluidic in Biomedical Applications
- Key applications of millifluidic in biomedicine
- Organ-on-chip models
- Millifluidic Organ On Chip Benefits
- Increased Mass Transport
- More Accurate Drug Diffusion
- Accelerated Cell Differentiation
- Technical Information:
- Examples of commercially available millifluidic platforms